The topics addressed and contents of this paper were written based upon student-submitted papers for the GNSS Accuracy Assignment, as part of the AGT 1007 Electronics and Control Systems course, fall semester 2020.
How did we get here?
In today’s precision agriculture world, GPS is at the core of many of the most common applications of this technology. From machine guidance and auto-steering, to variable rate application of inputs based on the location within the field, to mapping harvest yields, GPS is vital to achieving the desired outcomes of utilizing these systems.
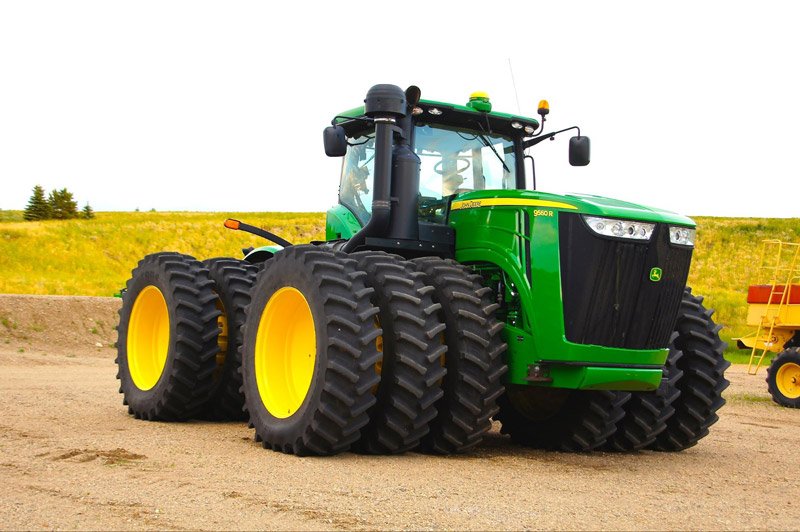
Figure 1: John Deere tractor with StarFire GNSS Antenna
Source: Madereugeneandrew (https://commons.wikimedia.org/wiki/File:John_Deere,_9560R.jpg), https://creativecommons.org/licenses/by-sa/4.0/legalcode
So what is GPS? GPS stands for Global Positioning System, and refers to the constellation of GPS satellites launched by the United States Department of Defence that began in 1978. By 1993, the complete GPS satellite system, consisting of 24 satellites became fully operational1. Today there are 28 operational satellites in the GPS constellation2.The initial purpose of GPS was to provide the US military with a robust navigation system that would allow personnel to know their position virtually anywhere on the planet, and the most accurate satellite signals were reserved for US military users only. Since 2000, civilian users also have access to these higher accuracy signals, and today’s dual frequency GPS receivers (as found in most current precision agriculture hardware) make use of both the L1 and L2 satellite bandwidths.
GPS isn’t the only satellite constellation today however. In fact, there are multiple additional constellations, many of which also provide global coverage. GLONASS, the Russian satellite navigation constellation is perhaps the most well known here in Canada because it provides increased satellites in view in the northern skies. This has greatly benefitted many agricultural users in northern latitudes that used to experience down-time when not enough GPS satellites were available for high accuracy position calculations. Notable others include BeiDou, the new Chinese constellation, and Galileo from the European Space Agency, both of which also provide global coverage. The term GNSS (Global Navigation Satellite System) refers to all of these constellations, not just GPS, and therefore today’s modern receivers should be referred to as GNSS receivers rather than GPS receivers, because they are capable of utilizing satellite signals from multiple constellations.
What makes GNSS accurate (or inaccurate)?
GNSS accuracy is an important consideration for all agricultural users. For some tasks, high accuracy may be less important, but for others it may be critical. Agricultural users therefore need to determine the acceptable accuracy level(s) for their requirements. Often this is a balancing act between cost and performance to achieve the optimum return on investment for a given application. For example a tractor used primarily for tillage may not require such high accuracy GNSS input into an auto-steering system as a tractor pulling a seeder that will perform inter-row seeding (placing seed accurately between the stubble rows left by the previous year’s crop).
GNSS receivers compute their position by measuring how long it takes for signals from different satellites to reach the receiver. Each satellite signal contains a message that tells the receiver exactly when the signal was broadcast by the satellite, as well as the position of the satellite in orbit at the time of transmission. Using a complex mathematical modeling process, the GNSS receiver attempts to compute the distance between it and the satellite based on the time taken to receive the signal. Modern dual frequency GNSS receivers repeat this process a second time as they also receive a second signal from each satellite. Once a minimum of 4 satellite signals are being received, the receiver can compute it’s position on the earth based on triangulation of it’s position in relation to each satellite.
The accuracy of GNSS is affected by numerous factors, the largest of which is atmospheric interference, as signals pass through space and into the earth’s atmosphere. An example of how the atmosphere can disrupt and delay satellite signals from different satellites is shown in Figure 2. Therefore, without accounting for these errors, we would see significant inaccuracies in the computed positions. While dual frequency receivers are able to account for some of the error by comparing the time taken for two signals on two different wavelengths to be received, they still cannot achieve the kinds of accuracies often needed for precision agriculture applications.